On September 14, 2015, the Earth was shocked by a cosmic tremble—an event that, however momentous, went all but unnoticed. Luckily, scientists at LIGO, the Laser Interferometer Gravitational-wave Observatory, were paying attention, and they quickly jumped to work to figure out what had happened. It took five months of thorough analysis until they could be sure that they had witnessed something historic. They were finally ready to spread the word: the Earth had indeed shaken, very subtly, as it was rocked by a passing gravitational wave. What is more, humans had for the first time directly detected this famously elusive phenomenon—wrinkles in the fabric of space and time, first predicted by Albert Einstein in 1916. “We DID it!” declared Caltech’s own David Reitze as he revealed the news during a press conference in Washington, D.C. This moment was so thrilling that the memory still gives me goosebumps; it is not every day that one gets to see history being made up close.
The LIGO detectors are exquisitely engineered machines designed to detect gravitational waves. There are two such instruments: one in Livingston, LA, the other in Hanford, WA. The main component is a powerful laser, whose light travels inside vacuum tubes protected by a concrete enclosing. This enclosing can be seen in the aerial pictures above. Each of the arms is 4 km (2.5 mi) long. Virgo, an instrument similar to LIGO, is located near Pisa, in Italy.
Caltech/MIT/LIGO Lab
Earth shakes all the time. Most of this agitation, however, is due to mundane reasons: wind, construction, and earthquakes, for example. Gravitational waves are different. Like ripples on the surface of a pond or sound waves through the air, gravitational waves travel through space carrying energy and information about their source. Unlike water or sound waves, however, gravitational waves do not need a material medium in which to move. Instead of pushing water or air molecules around, gravitational waves directly stretch and squeeze distances between objects by altering space itself. Rather than being created by a stone dropped in a pond or a car honking its horn, gravitational waves originate in the most violent events in the universe, like exploding stars or colliding black holes. After journeying over intergalactic distances for millions of years, the gravitational echoes of such cosmic cataclysms wash over Earth, allowing us to learn about the objects that created them. As such, gravitational waves are both a wonder of physics and a revolutionary tool for astronomy.
In a sense, gravitational waves are to gravity what light waves are to electromagnetism. Both are direct manifestations of one of the four fundamental forces (gravity, electromagnetism and the strong and weak nuclear forces), and carry in their nature invaluable clues about the fundamental structure of the Universe. The similarities are also observable; both kinds of waves travel at the same speed through empty space (670 million miles per hour) and are created by the acceleration of some type of charge (electric charge for light; gravitational charge, i.e. mass, for gravitational waves).
According to Einstein, massive bodies curve spacetime, altering the trajectories of nearby objects. In this language, we explain the motion of the Earth around the Sun not as a consequence of an instantaneous attraction between them (like Newton did), but as a consequence of the Earth moving in a straight line defined in spacetime curved due to the Sun. The green grid in the figure is a representation of this curvature (what physicists call an “embedding diagram”). In this picture, you can (approximately) think of the Earth moving around the Sun like a marble rolling around a bowling ball on the surface of a trampoline.
T. Pyle/Caltech/MIT/LIGO Lab
But the analogy with light can only take us so far. To gain a better appreciation of what gravitational waves are, and why their discovery was so important, we must re-examine our intuitions about space and time. If you are anything like me, the word “space” conjures up images of graph paper, a rigid grid that helps locate objects and events; on the other hand, “time” evokes a universal clock, ticking relentlessly towards the future. In this Newtonian picture, space is the stage on which the symphony of the Universe is played, and time is the metronome that keeps its tempo—both are essential for the performance to take place but are unaltered by the music.
As familiar as that picture may be, more than a century of experiments and intellectual inquiry have taught us that Nature simply does not work that way. They reveal instead that space and time are woven together into the malleable, four-dimensional fabric that we call “spacetime.” Spacetime is not a rigid canvas: it can bend, twist and curl. Spacetime is not passive: it can pull, push and stretch. In the symphony of the Universe, the stage and the metronomes dance to the music, and in turn influence the way it is played. This is the dynamic, nonlinear picture that we inherit from Einstein’s general theory of relativity—a stark contrast with Newton’s rigid, albeit more intuitive, paradigm.
As the eminent physicist John A. Wheeler put it, “spacetime tells matter how to move; matter tells spacetime how to curve.” Gravity is the observable effect of this curvature: Earth orbits the Sun because the spacetime on which the planet moves has been curved by the star. This is similar to the way a marble can be made to roll around a bowling ball lying on a trampoline. The analogy can be stretched a bit further: if we quickly shook our imaginary bowling ball, we would produce ripples propagating outward in the fabric of the trampoline; by the same token, if we could quickly shake the Sun, we would produce ripples propagating outward in the fabric of space. These wrinkles in the curvature of spacetime (aka “gravitational field”) are what we call gravitational waves.
Gravitational waves manifest themselves by changing lengths1, or more concretely, stretching and squeezing space on a plane transversal to the direction in which they move. This is a very real physical effect; were it strong enough, the strain would tear objects (and you!) apart. It is also a universal phenomenon, caused by almost any accelerated configuration of mass. As a result, the space around us subtly roils as it is shaken by gravitational radiation reaching us from sources far and close. To an extent, you are being wiggled by them right now.
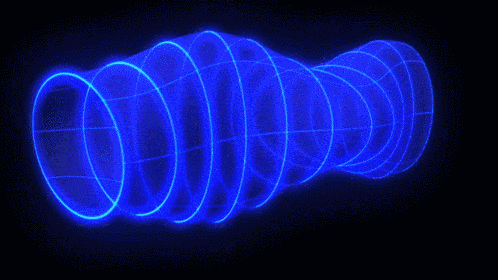
Imagine a series of circular rings floating out in space with their centers aligned so that they define a cylinder. A gravitational wave traveling along the axis of the cylinder will stretch and squeeze the rings, deforming them as in the animation.
ESA-C. Carrerou
It is natural, then, to wonder: if these waves are so pervasive, and their effect so obvious, why do they go unnoticed? The answer is that they are unimaginably weak. In a sense, spacetime is very stiff; it takes extremely violent events to bend it to any noticeable degree and, even then, the effect is miniscule. Detecting gravitational waves is a herculean task, and the effort to do so was long dismissed by many as little more than a pipe dream. It took decades of hard work by hundreds of physicists and engineers around the world to prove that it was possible.
One of the results of that global effort was LIGO. Its two instruments, one in Hanford, Washington, the other in Livingston, Louisiana, are extremely sophisticated machines that can measure the imperceptible displacements caused by a passing gravitational wave. To achieve this, a laser beam is split into two by a semi-transparent mirror (“beam splitter”), which redirects the light down two perpendicular, 4 km-long arms; at the end of each arm, the laser is reflected back to the beam splitter, recombined and sent out for readout. As a gravitational wave goes by, it effectively changes the relative length of the two arms. This modifies the distance traveled by light in the instrument. Thanks to exquisite optical techniques, this information can be used to measure displacements orders of magnitude smaller than the wavelength of the laser light. Advanced isolation from vibrations and vacuum technology make this possible even in spite of the machine’s noisy environment (wind, earthquakes, and human activity, to name a few offenders).
A simplified depiction of the LIGO instrument. A laser beam of light is generated and directed toward a beam splitter, which splits it into two separate and equal beams. The light beams then travel perpendicularly to a distant mirror. The mirrors reflect the light back to the beam splitter, repeating this process 200 times. When gravitational waves pass through this device, they cause the length of the two arms to alternately stretch and squeeze by infinitesimal amounts, tremendously exaggerated here for visibility. This movement causes the light beam that hits the detector to flicker.
T. Pyle, Caltech/MIT/Ligo Lab
At its peak, the first gravitational wave LIGO detected (nicknamed GW150914) changed the lengths of LIGO’s 4 km arms by a distance roughly one thousand times smaller than the radius of a proton (around 10-18 m). By thoroughly analyzing the signal, which lasted around a quarter of a second, we learned it originated in the collision of two black holes over 1 billion light-years away. As the two objects, each weighing more than 30 times the mass of our Sun, plunged towards each other, they rattled the spacetime around them and, in their final moments, released more power than all of the stars in our Universe combined. But the violent collision was invisible; all that energy was emitted solely in gravitational waves. The ancient echo of this cosmic crash eventually reached Earth and was observed by LIGO’s instruments, shortly after they had been turned on for the first time. This was not only the first direct detection of gravitational waves, but also of pairs of black holes—mysterious objects that we are still struggling to understand. As a result of this discovery, three of LIGO’s founders, (Rai Weiss from MIT, Kip Thorne and Barry Barish from Caltech) received the 2017 Nobel prize in Physics.
A supercomputer simulation shows the collision and merger of two black holes, a tremendously powerful event detected for the first time by LIGO. This simulation shows how the merger would appear to our eyes if we could somehow travel in a spaceship for a closer look. It was created by solving equations from Albert Einstein's general theory of relativity, informed by the LIGO data.
Simulating eXtreme Spacetimes (SXS)
The detection of GW150914 was only the beginning; since then, over ten more events like it have been observed. Most remarkably, in August of 2017, LIGO and its European counterpart, Virgo, made the first observation of the merger of two neutron stars (GW170817). Neutron stars are one of the possible remains left by exploding stars, and pack roughly the mass of our Sun into a sphere the size of Caltech’s home city of Pasadena. Their collisions are catalysts for very important physical processes, including forging heavy metals like gold and platinum. (The astronomer Carl Sagan liked to remind us that we are stardust; but, as my thesis advisor, Alan Weinstein, likes to say, our jewelry is neutron-star dust!)
We can convert the data recorded by the LIGO detectors into sound. This video shows the result for our first signal (GW150914). The x-axis represents time, while the y-axis represents the frequency (pitch) of the signal; the brightness of the color indicates the frequencies in which the signal was concentrated at any given time (brighter meaning more energy). The top and bottom panels show the data for the Hanford and Livingston detectors respectively. In the first two runs of the animation, the sound-wave frequencies exactly match the frequencies of the gravitational waves as detected by our instruments. The second two runs of the animation play the sounds again at higher frequencies that better fit the human hearing range. As the black holes spiral closer and closer together, the frequency and amplitude of the gravitational waves increase, yielding a characteristic 'chirp.'
Caltech/MIT/Ligo Lab
In spite of their exceptional scientific richness, our discoveries so far represent only a sliver of what can be potentially learned about the physics of the cosmos with gravitational waves. In the upcoming years, existing gravitational-wave detectors will be improved to achieve their maximum sensitivity, and will be joined by new observatories in India and Japan. These ground-based instruments will subsequently be complemented in space by LISA, a joint European Space Agency and NASA mission which will be hunting for signals from merging massive black holes in the centers of distant galaxies, among other sources. All this, plus continued partnerships with electromagnetic and neutrino observatories, means that we will soon be inundated with an unprecedented wealth of data surely holding many fundamental clues about Nature.
Our exploration of the universe through gravitational waves has just begun. Every other time humanity has gained a new paradigm in which to study the world, the universe turned out to be more surprising and exciting than we ever imagined—this time will be no different.
Footnotes
1: This is not a full explanation of the effect and nature of gravitational waves: that would require a discussion of gauges and other technicalities beyond the scope of this article.
Further Reading
2: The basic physics of binary black hole mergers
3: Observation of merging neutron stars
4: LIGO and Virgo’s detections during the first two observation runs